Research Article, J Pharm Drug Deliv Res Vol: 10 Issue: 1
Hydrogel Dressings for Chronic Wound Healing in Diabetes: Beyond Hydration
Paul R Hartmeier1, Ngoc B Pham1, Ketki Y Velankar1, Fadi Issa2, Nick Giannoukakis3,4, and Wilson S Meng1,5*
1 Graduate School of Pharmaceutical Sciences, School of Pharmacy, Duquesne University, Pittsburgh, USA
2 Nuffield Department of Surgical Sciences, University of Oxford, John Radcliffe Hospital, Oxford, UK
3 Institute of Cellular Therapeutics, Allegheny Health Network, Pittsburgh, USA
4 Deparment of Biological Sciences, Carnegie Mellon University, Pittsburgh, USA
5 McGowan Institute for Regenerative Medicine, University of Pittsburgh, USA
*Corresponding Author : Hartmeier PR
Graduate School of Pharmaceutical Sciences, School of Pharmacy, Duquesne University, Pittsburgh, PA 152, USA
Tel: 4123966366
E-mail: meng@duq.edu
Received date: December 1, 2020; Accepted date: December 14, 2020; Published date: December 21, 2020
Citation: Paul RH, Ngoc BP, Ketki YV, Fadi I, Nick G, et al. (2020) Hydrogel Dressings for Chronic Wound Healing in Diabetes: Beyond Hydration. J Pharm Drug Deliv Res 10:1.
Abstract
Chronic wounds caused by diabetes are a significant medical challenge. Complications from non-healing can result in dire consequences for patients and cost the healthcare system billions of dollars annually. Non-healing in wounds for diabetic patient’s results from a combination of factors which impair clearing of injured tissue, proliferation of healthy cell populations and increase risk of infection. Wound dressings continue to form the basis for the treatment of chronic wounds. Traditionally, these focused solely on hydration of the wound site and mitigating infection risk. Hydrogel systems are ready made to meet these basic requirements due to their intrinsic hydration properties and ability to deliver active ingredients. Flexibility in materials and methods of release allowed these
systems to remain targets of research into the 21st century. Improved understanding of the wound environment and healing cascades has led to the development of more advanced systems which incorporate endogenous growth factors and living cells. Despite their promise, clinical efficacy of these systems has remained a challenge. Further, the regulatory pathways for approval add a layer of complexity to translate pre-clinical work into marketed products. In this review, we
discuss systems currently in clinical use, pre-clinical directions and regulatory challenges for hydrogels in the treatment of diabetic chronic wounds.
Keywords: Diabetes; Chronic wounds; Hydrogels; Drug delivery; Self-assembling peptides
Introduction
Diabetes remains a major health challenge across the United States with approximately 27 million people affected in 2018 [1]. Chronic ulcers and impaired wound healing that result from diabetic neuropathy and vasculopathy are common and associated with potentially severe consequences, including limb loss. An analysis of 1381 patients determined a 4.96% (3.1%-6.82%) rate of foot ulcers 19 years after diagnosis of type II diabetes in the United States [2]. Globally, incidences of diabetic foot ulcers for type I and type II diabetes were 5.5% (3.2%-7.7%) and 6.4% (4.6%-8.1%) [3], respectively. In the US, between 2005 and 2010, 33% of diabetesrelated hospital admissions resulted from healing impairment and rates were 10 times those of non-diabetic patients [4]. Treatment for ulcers and associated complications places a large burden on the healthcare system with costs of ulcer treatments in excess of a billion dollars in 2012 [4]. Treatments for these complex wounds are constantly evolving but dressings continue to be the mainstay. For decades, polymeric biomaterials have formed the backbone of dressings and therapeutic delivery platforms in the management and treatment of diabetic chronic wounds.
Ideal wound dressings are designed to meet the following functions: humidity retention, gas exchange, prevention of microorganism growth, thermal insulation, biocompatibility, mechanical strength, sterility, ease of removal, and cost-effectiveness. Beyond these basic considerations, modern dressings are designed to absorb exudate, assist cell adherence and proliferation, and deliver therapeutic agents such as antimicrobials or growth factors [5-8]. In this review, we discuss different dressing systems, with a focus on hydrogel platforms, for use in diabetic chronic wounds.Ideal wound dressings are designed to meet the following functions: humidity retention, gas exchange, prevention of microorganism growth, thermal insulation, biocompatibility, mechanical strength, sterility, ease of removal, and cost-effectiveness. Beyond these basic considerations, modern dressings are designed to absorb exudate, assist cell adherence and proliferation, and deliver therapeutic agents such as antimicrobials or growth factors [5-8]. In this review, we discuss different dressing systems, with a focus on hydrogel platforms, for use in diabetic chronic wounds.
Challenges in Diabetic Wound Healing
Skin anatomy
Development of wound dressings relies on physiological knowledge of human skin which is histologically comprised of epidermis, dermis and hypodermis, each with its own unique composition, function, and developmental origin [9,10]. The epidermis is the outermost layer and contains keratinocytes, melanocytes, Langerhans cells and Merkel cells distributed across four sub-layers: basal, squamous, granular, and cornified. Keratinocytes are responsible for ‘waterproofing’ and wound sealing. Under normal condition in humans, it takes 28 days for keratinocytes to mitotically divide at the basal sublayer and gradually migrate to the outer sublayers of the epidermis. The dermis contains vascular components, adnexal structures such as nerve endings, glands, and hair follicles. The structural components are formed of an extrafibrillar matrix, types I and III collagen, and elastic fibers. The three principal cell types of the dermis are macrophages, mast cells, and fibroblasts, with the latter being key players in dermal wound healing. The hypodermis consists of connective and adipose tissues.
Wound healing in healthy individuals
Acute wound healing in healthy individuals proceeds through four phases: hemostasis, inflammation, proliferation and maturation, and remodeling [11,12]. Hemorrhaging is the first event upon injury and aides in pathogen elimination. Fibrinogen in the blood will coagulate and induce hemostasis. Simultaneously, neutrophils and macrophages infiltrate the wound site to engulf pathogens and dead cells as well as producing growth factors. The inflammatory phase begins within minutes post-injury and lasts approximately three days. In the proliferative phase, the wound is restored through fibroblast proliferation, Extracellular Matrix (ECM) reorganization, angiogenesis, collagen synthesis, granulation tissue formation and epithelization [12]. The final remodeling phase is characterized by further epithelization, strengthening of new tissues and maturation of granulation tissues into acellular scars.
Chronic wounds and impact of diabetes
Chronic wounds are characterized as not progressing from the inflammatory stage of healing [13]. Remaining in this stage can lead to complications such as infection, neoplastic transformation, necrosis and potential amputation. Development of chronic wounds in diabetes has been linked to a myriad of factors including impairment of growth factors, interruption of angiogenesis, reduced immune function, and decreased cell migration, accumulation of ECM components and imbalance of matrix metalloproteases [14,15]. Hyperglycemia in diabetic wounds induces high amounts of reactive oxygen species which, among other consequences, increases expression of proinflammatory cytokines such as TNF-α [13,16]. Infections in the wound bed exacerbate the inflammation through an upregulation of reactive oxygen species from the host immune response and bacterial excretion. The overexpression leads to an inflammatory feedback loop and apoptosis of endothelial cells [13,17,18].
Endothelial progenitor cell migration from the bone marrow is a critical step in regeneration and is well-documented to be impaired in type 1 and 2 diabetes [19]. Impeded endothelial progenitor cell proliferation from bone marrow results from a decrease in glucosemediated epithelial nitric oxide synthetase at the site of injury; prompting a reduced circulation of endothelial progenitor cells. Compounding lack of proliferation from bone marrow is reduced SDF-α expression near the wound which hinders endothelial progenitor cell homing [14,19]. Differentiation and proliferation of endothelial progenitor cell are further impacted by hypoxia and hyperglycemia induced reduction of growth factors such as Vascular Epithelial Growth Factor (VEGF) and Fibroblast Growth Factor (FGF) [13,17,20]. Chronic ulcers have high levels of matrix metalloproteases, resulting from high levels of reactive oxygen species, damage restructuring of the epidermis through degradation of growth factors and structural proteins such as collagen [15-17,19]. Chronic wound healing can be enhanced through wound hydration, pH balance and providing scaffolding for the migration of cells [6].
General Dressings for Diabetic Wounds
Antimicrobial dressings
A moist, bacterial-free environment is ideal for wound healing [21,22]. An appropriate moisture level prevents cell dehydration, enhances angiogenesis, and fosters dead cell degradation [23]. Under moist dressing conditions, epithelial and dermal cells were observed to proliferate faster, while inflammatory cell expansion reduced [24]. In the following narrative, we will focus on wound dressing systems which provide moisture balance for optimal wound healing.
Infection control is a priority in managing diabetic wounds because hyperglycemia and reduced immunity promote bacterial proliferation [14,15,25]. For example, isolates of Staphylococcus aeneous from human diabetic foot ulcers demonstrated a phenotypic change to less virulent subtypes, implying the barriers to infection were reduced [26]. To mitigate infection, silver dressings are often utilized for their antimicrobial properties. Upon exposure to exudate or fluid, silver is activated and engages several cellular targets on both gram positive, Gram negative bacteria and fungi [27]. In the case of a low exudate level, the dressing can be briefly conditioned with water before application.
Acticoat (Smith & Nephew, UK) is a popular representative of silver-incorporated wound dressings. The dressing has a polyester/ rayon core and an outer nano-crystalline silver coated High-Density Polyethylene (HDPE) mesh [28]. Rapid release of silver within 30 minutes makes Acticoat suitable as an initial dressing strategy [29,30]. Acticoat can remain intact on wounds for three to seven days depending on the product version and the quantity of exudate. Despite successful reports for the treatment of diabetic foot ulcers, Acticoat and similar dressings present considerable limitations [31]. In dry wounds, it is common to rinse wounds with normal saline, but chloride can interfere with the antimicrobial effect of silver. Another complication is that prolonged silver exposure can hinder proliferation of fibroblast and keratinocytes and delay healing, and also result in adverse scarring. Nanocrystalline dressings are also expensive, making their use in the treatment of chronic wounds uneconomical.
Hydrocolloid dressings
Due to the compromised healing capacity of diabetic patients, wounds often rapidly ulcerate [32]. Thus, hydrocolloid dressings are commonly used for their exudate absorption property [8,33,34]. These dressings typically consist of a colloidal absorbent layer that gels upon exposure to moisture and backed with a film layer or foam. The absorbent must be in contact with exudate to form a cohesive gel layer, which then absorbs exudate, traps microorganism or cell debris, and allows gas exchange [6,8,35]. Common colloidal materials are carboxymethylcellulose, gelatin, pectin, and actin [11]. Because hydrocolloids can dissolve rapidly in excess exudate, water-insoluble elastomers are often included to improve their elasticity and mechanical properties [34]. The adsorption of the exudate enables the wound to remain hydrated while protecting the underlying tissues from sheer stress and contamination [5,8].
Duoderm (ConvaTec Ld, UK) is a representative hydrocolloidal dressing approved for diabetic foot ulcers [33,36]. The composition Duoderm includes pectin, gelatin, and carboxymethylcellulose. Pectin is a biodegradable, biologically inert hydrocolloid made up of linear chains of plant galacturonic acid that gels upon esterification [36]. Gelatin, a hydrocolloid derivative of collagen, forms a nanofiber network that resembles the extracellular matrix, with high porosity and large surface area [37]. Carboxymethylcellulose is highly absorbent, allowing exudate removal into the dressing. The combined properties of these polymeric materials render a product with strong adhesion yet allowing aeration and diffusion of nutrients.
The use of hydrocolloid dressings in diabetic wounds is controversial [5,6,8]. Extended use of hydrocolloid dressings can lead to malodorous gel, increased risks of infection, maceration of the wound edge, and contact dermatitis [35]. Insufficient gas exchange may lead to hypoxia, which exacerbates inflammation and cellular damage [14]. Prolonged adhesion of hydrocolloids can result in secondary tissue damage during removal [38]. Thus, hydrocolloids are more suitable for low to moderate exuding wounds and must be changed every seven days or less [34,38].
Hydrogel dressings for diabetic wounds
An overview of hydrogel functions in the wound environment if provided in (Figure 1) Hydrogels are commonly used for the management of chronic wounds because of their occlusive property by which moisture can be maintained [6,8,39]. Compared to fibrous or hydrocolloid dressings, hydrogels can rehydrate necrotic and sloughy wounds and are suitable for wounds with low or medium exudate [40]. Resembling the structural consistency of natural ECM, hydrogels can function as instructive scaffolds for keratinocyte proliferation and angiogenesis [41,42]. Their hydrophilic and semi-solid nature is conducive for easy application and removal while providing a cooling effect. Another feature is that hydrogels are semi-transparent insofar wound monitoring is possible between dressing changes [43]. These recognized advantages have made hydrogels attractive as dressing materials.
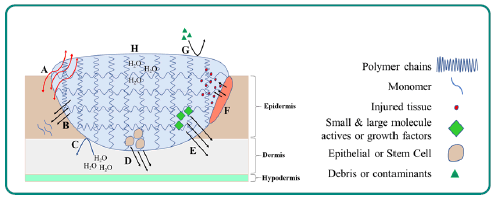
Figure 1: Overview of Hydrogel Function in the Wound Environment. A: Semi-permeable nature of hydrogels allows for transfer of gasses and aids in oxygenating wound sites to reduce hypoxia. B: Bioactive polymers (i.e. hyaluronic acid) delivered to wound site as cross-linked polymers degrade into lower molecular weight polymers and monomers. C: Swollen hydrogels retain water and prevent dehydration of wound site. D: Hyperglycemia results in apoptosis of endothelial cells; epithelial, STEM and other cell varieties loaded into gel migrate to wound site to replace cell populations and aid in re-epithelization. E: Reduced immune function in diabetics increases infection risk and hyperglycemia decreases available endogenous growth factors. Delivery of small and large molecule actives, as well as growth factors, manage infection and promote healthy cell proliferation. F: Swollen gels aid in wound debridement. G: Gel layer protects wound from debris and other contaminants. H: Polymer chains cross-link to form matrix which entraps and retains moisture.
Hydrogels are cross-linking polymers interweaving into water-filled 3-dimensional matrices. The assembly can occur through covalent, ionic, and/or hydrophobic interactions of polymer backbone and side chain atoms [44]. The degree of hydration, measured as swelling ratio in percent weight change, is a primary characteristic of hydrogels with typical ranges between 70%-99% w/w [45]. The change in hydrated mass in sol-gel transition is a critical manufacturing quality attribute. The extent of cross linking will influence the stiffness of the gel, with softer gels being more susceptible to removal through sheer. Polymer composition can be tuned to a specific stiffness, typically ranging from 0.5-5.0 MPa [5,44]. Defective cross-linking causes reduced swelling capacity, resulting in insufficient hydration of the wound site as well as limiting exudate absorption [39,46]. In addition, insufficient crosslinking increases degradation via increased polymer chain mobility and exposure of cleavable groups [47,48].
Natural and endogenous polymeric hydrogels generally exhibit good biocompatibility, with low risk of inducing acute or chronic inflammation or immunological reactions [49]. Commonly utilized polymers include collagen, chitosan, cellulose, gelatin, hyaluronic acid, silk fibroin, etc [6,50]. Of note is hyaluronic acid, which is naturally present during wound healing, promotes angiogenesis, enhances fibroblast proliferation and reduces reactive oxygen species damage [6,8]. In diabetic rats, hydrogels containing high or low molecular weight hyaluronic acid significantly improved healing time from approximately 70 days to 50 days [51,52]. Additionally, reduced CD45+ expression and increased VEGF expression at days 3 and 10 in the hyaluronic acid treated groups indicated a reduced inflammation and an increased angiogenesis, respectively. Similarly, a gelatin/ hyaluronic acid hydrogel showed improved wound closure in diabetic mice when loaded with thrombodulin, which stimulates growth of keratinocytes [53]. The high-swelling hydrogel was capable of swelling to 11-fold weight percent and sustained release of thrombodulin over 12 hours. Several hyaluronic acid-based products are approved by the FDA for use in wound healing. HyalofilTM, an esterified hyaluronic acid -based hydrogel with up to 3000% swelling potential, exhibited healing in ulcers from 1 in 9 (control) to 10 of 13 (active) with significance [54]. HyGelTM is another hyaluronic acid - based hydrogel approved for the treatment of chronic and diabetic wounds [55]. Several other hyaluronic acid-based products are approved for diabetic foot ulcers and hyaluronic acid continues to be used in emerging pre-clinical works.
Collagen, as an ECM component, also offers advantages for wound healing. Collagen-based hydrogels significantly reduce wound area at 28 days in diabetic mice relative to a control group and an Endothelial Growth Factor (EGF) loaded gel [56]. In the same study, collagenbased gels exhibited an increased fibroblast proliferation after 4 weeks relative to EGF loaded gels. Collagen-based hydrogels are present in several approved products. Collatek®, for example, contains collagen cross-linked with polyacrylic acid and was approved in 2002 for diabetic foot ulcers.
A caveat of natural polymeric hydrogels is mechanical strength. Incorporating synthetic polymers such as Polyvinyl Alcohol (PVA), Poly(Ethylene) Glycol (PEG), polyacrylamide, and polylactic acid, can improve the hydrogel physical properties [50]. Polyesters, such as Poly Lactic-Co-Glycolic Acid (PLGA), and polyurethanes have favorable degradation properties and form hydrogels in conjunction with hydrophilic polymers such as polyvinyl-pyrrolidone [6,8,57].
Carboxymethylcellulose is commonly found in hydrogels. IntrasiteTM is a carboxymethylcellulose -based gel which has been approved for the treatment of diabetic wounds. Autolytic debridement is a main feature of this dressing and was reported in several studies [58]. Specifically, the hydrophilic polymer cross-linking expands to absorb the exudate and trap dead cell debris without damaging the fragile granulation tissues [22,43]. Because the dressing can help clear dead cells, the inflammatory phase can be shortened and thereby not affect the cellular activities in later phases of healing. The product is thought to be beneficial for granulation and re-epithelization at the proliferative and remodeling stages [59]. The hydrophilic content of this dressing facilitates the migration of epithelization cells. The pH of open wounds is typically more than 7.1, but IntrasiteTM dressing can reduce the environment pH to around 6.8, which is ideal for fibroblasts to produce collagen [58,60].
Due to their porosity, hydrogels are suitable for incorporating therapeutic agents, wound filling materials and instructive nanostructures [44,61]. At the swollen state, the pore size in the hydrogel can be designed to be large enough (in the low hundred μm range) for diffusion of bioactive molecules but small enough to prevent bacteria from accessing the wound. Vascularization has been observed across the gel matrix with pore sizes greater than 50 μm but is limited to the surface in smaller pore sizes [62].
pH responsive polymers have proven advantageous for diabetic wounds because the wound pH changes significantly throughout the healing process. Increased swelling potential upon pH changes promotes a more consistent wound environment [45,63,64]. Thermoresponsive hydrogels with gel transitions near body temperature offer advantages by remaining as low viscosity liquids until application. Recent advents include devices placed near the gel to modulate temperature for drug release from the liquid phase or retain drug in the gel phase [65].
Hydrogel dressings for microbial control
Consistent with fibrous and hydrocolloid dressings, hydrogels impregnated with silver have been developed for use in chronic wounds. SilverMed AgTM (MPM Medical), Silver SeptTM (Anacapa Technologies), and Viniferamine® (Viniferamine) are three representative products on the market incorporating low levels (<0.01%) of silver (MPMmed, Anacapa and Viniferamine). Silver SeptTM exhibited 99% elimination of S. aureus in-vitro after 1-day post-exposure and has improved outcomes in clinical case studies (Anacapa). In addition to dissolved silver, silver nanoparticles have been incorporated into hydrogels. SilvrSTAT®, for example, reduces S. Aureus greater than 99% within 1 hour in-vitro [66]. Incorporation of nanoparticles allows for more controlled release from hydrogels but may impact bulk physical properties. Swelling capacity of chitosan- PEG based hydrogels decreases by up to three times when loaded with silver nanoparticles [67]. This change is not accompanied by reduction in porosity and may be attributed to increase cross linking via nanoparticle binding with polymer functional groups [67,68]. Silver has also been used as a cross-linking agent through a coordination with thiolated-PEG. The resulting hydrogel exhibits favorable mechanical properties with elastic and loss moduli capable of withstanding up to 50% increase in strain. This elastic recovery is preferable for an injectable or topical product and S. aureus density has been shown to reduce 3x relative to the control [69].
Sustained release of small molecule antibiotics from hydrogels has also been reported. Tetracycline hydrochloride loaded into gelatin microspheres exhibited 50% release over 48 hours from an alginate/ carboxymethyl chitosan hydrogel. This system exhibited a 2-log decrease in S. aureus after 24 hours compared to the gel alone [70]. Likewise, gentamicin formulated into poly(N-isopropylacrylamide-codimethyl- γ-butyrolactone acrylate-co-Jeffamine® M-1000 acrylamide) hydrogel has been shown to continue to be released up to 7 days in rabbit models and completely eradicates induced infection with S. aureus [71]. Gentamicin has also been co-formulated with Zinc-Oxide (ZnO) in a chitosan-based hydrogel to provide a synergistic antibiotic effect. The gel exhibits a swelling ratio of 150:1 and inhibits growth of S. Aureus in-vitro with a minimum effective concentration of less than 0.5 μg/ml [72,73]. A variety of other antimicrobials including, gold, copper, iodine and other small molecule antibiotics have been included in hydrogel dressings [73].
Hydrogels to Deliver Growth Factors, Biologics and Cells
Growth factors have been successfully delivered via hydrogels with success in pre-clinical and clinical settings. Among the growth factors, Platelet-Derived Growth Factor (PDGF), SDF-1, basic FGF (bFGF), EGF, VEGF, Transforming Growth Factor Beta (TGFB) are the most common[74]. These factors trigger epidermal cell and fibroblast proliferation, and angiogenesis. Many pre-clinical studies have been published on delivering growth factor with biomaterial scaffolds, hydrogels and nano-micro-particulate systems [75]. However, FDA approval of these drug-device combination products is challenging. Further complicating the approval landscape is limited success of growth factors as monotherapies in clinical trials. The first and only FDA-approved dressing for diabetic ulcers utilizing growth factors is Regranex® Gel (Smith & Nephew). PDGF in the product promotes fibroblasts which increases granulation tissue, enhances reepithelization rates, revascularization and collagen synthesis. In clinical trials, complete healing of diabetic ulcers was observed in 50% of patients using Regranex® 0.01% relative to less than 30% using the placebo gel [76]. Despite this success, a single growth factor may be insufficient to improve healing across all patient populations [14,77-79].
SDF-1 expression is reduced in diabetic wounds causing lack of EPC migration and reduced vascularization [80]. Sustained release of SDF-1 over 48 hours has been achieved in a gelatin-based hydrogel and demonstrated in-vitro recruitment of MSCs and macrophages. Kim and Tabata [81] In this study, release of SDF-1 was further modified by the use of gelatin hydrogels with isoelectric points of 5 and 9; the gel with isoelectric point 9 exhibited a 20% higher burst release in normal saline. An extended release of SDF-1 was also observed using a thermo-responsive PEG Citrate-Co-NIsopropylacrylamide (PPCN) for up to 3 weeks after injection [82]. In diabetic mice, use of this SDF-1 PPCN reduced time to 50% wound healing improved to 11 days, compared to 14 and 16 days for neat PPCN and SDF-1 alone, respectively. In particular, the PPCN hydrogel system highlighted advances in hydrogel development by combining thermo-responsiveness and introducing antioxidant activity through design of the hydrogel [82,83]. The multifaceted impediments to healing in diabetic wounds will benefit from such approaches that target several pathways at once. Increased ROS is a major concern in diabetic wounds and the PPCN system is capable of scavenging 70% of radicals while thermo-responsiveness allowed for ease of application.
bFGF similarly would benefit from controlled delivery and has shown promise in pre-clinical wound healing. Sustained release of bFGF from gelatin microsphere hydrogels has been shown to be possible for up to 6 days using a hydrogel that remains stable for up to 30 days [84]. When applied to diabetic mouse wounds, epithelization and angiogenesis were significantly improved. At day 15, the epithelization rate of the bFGF loaded gel was 78% compared to 50% in the gel treated mouse alone. CD31+ cells were significantly higher as a percent of area under microscopic view (1.3%) compared to non bFGF treated wounds (0.4%), implying improvement in angiogenesis [85]. In a study examining bFGF and EGF loaded into a glycol chitosan hydrogel, 90% of the growth factors were released over 30 days in PBS after an initial burst release of 60% [86]. Swelling was not impacted by bFGF and EGF loading and in-vitro cell proliferation was significantly higher than bFGF or EGF alone. When assessed in non-diabetic mouse wounds, there was a 4 to 6-fold reduction in wound area after 7 days over the individual growth factors and neat gel. Although the system in this study was not applied directly to a diabetic model, other works have shown delivery of bFGF and EGF improves healing in diabetic mice. EGF and bFGF modified with lowmolecular weight protamine to increase penetration across the epidermis were loaded into a Carbopol gel. In diabetic mice, the growth factor loaded gels showed 90% wound area reduction after 12 days compared to 70% for the gel alone [87]. Another system has utilized FGF 21 in a heparin-poloxamer hydrogel in diabetic mice [88]. FGF 21 varies from bFGF in that it does not promote proliferation but is a metabolic regulator which upregulates glucose transporter GLUT 1; mitigating effects of hyperglycemia [89]. FGF21 loaded hydrogels improve wound closure rate to 93% in diabetic mice, compared to 83% when applied without loading. This suggests that FGF 21 delivery alone is less beneficial than with extended release. In this study, collagen deposition was significantly higher in both FGF 21 groups compared to non-FGF 21 controls, and FGF 21 loaded hydrogel was, again, higher than FGF 21 alone.
VEGF has been used in a variety of hydrogel systems in diabetic mice; however, to date it has not been efficacious as a monotherapy in clinical trials. For example, human VEGF does not show any clinical improvement over placebo for time to complete wound healing [78]. Cross-linked heparin matrices can form hydrogels and offer advantages for growth factor delivery. The strong binding of growth factors to heparin, through the highly negative charge on sulfate and carboxylate groups, allows for loading of growth factors but limits release kinetics [90]. VEGF release from a star-PEG crosslinked heparin hydrogel was increased 2 times through selective removal of heparin sulfate groups [91]. When used in diabetic mice, VEGF loaded heparin hydrogels significantly improved angiogenesis evidenced by doubling the area with CD 31+ endothelial cells. Desulfation was also important in reducing the anticoagulant activity of heparin to less than 1%, as anticoagulation would be detrimental to wound healing. A heparin-PVA hydrogel has also been used for extended release of co-formulated bFGF and VEGF [92]. Here, burst release of bFGF was reduced approximately 5x due to the high binding between bFGF and heparin. VEGF also showed reduced burst but to a lesser extent. Further, the heparin-PVA hydrogel did not exhibit any reduction in swelling capacity relative to the PVA gel. Invitro HUVEC migration showed a 50% increase in outgrowth for the duel loaded gel relative to the growth factors alone and a 7x increase over the PVA heparin gel alone [92]. These growth factors loaded systems address the lack of healing by promoting proliferation of endogenous cell populations, but exogenous cell populations also have potential for accelerated healing.
The effectiveness of acellular dressings, even those with incorporated growth factors, relies on migration and proliferation of the endogenous cell populations. Thus, newer strategies are designed to incorporate live stem cells, fibroblasts and keratinocytes into the dressings. The seeded cells are meant to act as precursors to attract more cells to the wound site leading to faster healing. The optimization of these cellular products is driven by the need to minimize immunogenicity of the delivered cells and to make the cellular scaffold absorbable after tissue recovery.
As noted above, hydrogels are favorable for their ability to mimic the ECM environment and compatibility with various cell types [41,93]. Mesenchymal stem cells are of particular interest for their ability to differentiate and excrete growth factors to promote wound closure and vascularization [94]. Hydrogels offer an advantage to seeding cells as they will retain the cells in an appropriate environment for a longer period, allowing for better differentiation. Mesenchymal stem cells seeded into a collagen-based hydrogel result in a significant reduction in wound healing time in non-diabetic mice relative to hydrogel without mesenchymal stem cells and mesenchymal stem cells seeded without hydrogel [93]. In another work, an n-isopropylacrylamide thermo-responsive hydrogel, with a 33°C transition temperature, was compatible with and retained mesenchymal stem cells. When the mesenchymal stem cell loaded gel was applied to diabetic mice, expression of bFGF and TGF-β1 in the wound area increased compared to the hydrogel alone; resulting in significantly reduced wound size [94].
Adipose derived stem cells have similar benefits to mesenchymal stem cells as targets for hydrogel delivery. A hyperbranched-PEG (HP-PEG) crosslinked with thiolated-HA hydrogel has been shown to be capable of in-situ gel formation with a storage modulus near that of soft tissue [95]. Adipose derived stem cells loaded into the HP-PEG/ thiolated-HA survived at least 7 days and excreted VEGF and TGF-β1 at levels comparable to plated samples grown under optimal conditions. In diabetic mice, HP-PEG/thiolated-HA hydrogels loaded with adipose derived stem cells significantly increased wound closure rates, with evidence for increased CD31+ positive vessels relative to blank gel and non-embedded adipose derived stem cells. In another study, residual wound area percent at 14 days in diabetic rats was significantly reduced with adipose derived stem cells loaded into a poloxamer based hydrogel relative to adipose derived stem cells or the gel alone [96]. Consistent with other works, CD31+ and mRNA expression for VEGF and TGF-β1 was significantly increased in the adipose derived stem cells loaded gels, indicating accelerated angiogenesis and matrix depositions.
Beyond stem cells, fibroblasts have been successfully delivered using a PVA based hydrogel. The hydrogel was modified with phenylboronic chitosan, and benzylaldehyde-capped PEG to be pH and glucose responsive. The storage and loss moduli were 100x higher in the presence of glucose and above pH7. Cell viability assays demonstrated compatibility with fibroblasts and exhibited higher proliferation in less cross-linked hydrogels. In diabetic mice, the fibroblast loaded hydrogels exhibit significant reduction in wound area % at each measured time point, up to 18 days, and higher CD31+ cells at 7 days [97]. Taken together, cell-loaded hydrogels have the potential to improve outcomes by reinforcing or replacing endogenous cell populations impacted by complications of diabetes.
Self-assembling peptide hydrogels
Recently, self-assembling peptide hydrogels have become focal points as delivery vehicles for actives and cells. Low molecular weight gelators form hydrogels under specific environmental conditions through mechanisms such as hydrogen bonding, metal chelation, pi-pi bonding van der Waals forces or hydrophobic bonding [98]. Selfassembling peptides are a class of low molecular weight gelators which form supramolecular structures most commonly through β- sheet interactions with tunable hydration, mechanical and degradation properties based on the peptide sequences to provide numerous benefits for use in wound healing applications [98,99]. While this class of hydrogels has yet to be applied extensively to diabetic wounds, they have shown promise in healing through delivery of growth factors and immune modulators. RADA16 was the first commercially available self-assembling peptide for hydrogel formation and has been used in the delivery of bFGF for bone repair [100]. The highly adjustable nature of SAPs allows for molecular handles to be fused to retain biologics such as antibodies or growth factors. Fusion of Z15 to an EAK SAP, for example, provides an Fc binding domain allowing for sustained antibody release up to 28 days [101,102].
Wound healing models
Pre-clinical studies rely on in-vitro and in-vivo animal models and it is important to note the limitations of these systems. Common in-vitro models include monoculture and co-culture cell cultures in which a single cell type or multiple cell types, respectively, are examined. Wounds are modeled by scratching a section of the cells and observing the regrowth into the affected area [103]. Both methods are advantageous because of the relatively low cost, rapidity, and ease of sampling. Compared to monoculture, co-culture offers more complexity in simulating human wounds; however, the major limitation of both methods is the oversimplification of in-vivo wound environments. Typically, both studies employ keratinocytes and fibroblasts but cannot probe the interactions from other epithelial cell types, immune cells nerves and other cell types present in human skin [103]. To gain an additional layer of complexity, ex-vivo studies can be used to provide a more accurate representation of the in-vivo skin environment. The major advantage of ex-vivo studies is the 3D nature of the system and a more representative cell population beyond keratinocytes and fibroblasts. In addition, other environmental factors such as pH, nutrient transport and temperature can be more easily mimicked in this system [104]. Like cell cultures however, they are still limited in that they lack innervation from nerves and are less standardized than cell cultures alone [105].
Animal models can offer a more accurate representation of the skin environment but differences in skin anatomy between animal and human skin are a major concern [105]. Rodents, for example, lack apocrine sweat glands, have looser and more flexible skin which is dependent on the hair cycle. For wound healing studies, another major consideration is the presences of subcutaneous muscles, panniculosus carnosus, which persist throughout the entire skin of the while in humans are only present in select regions [106]. These muscles change how the skin heals and in-contrast to human wounds, which heal primarily through re-epithelization, murine wounds heal through skin contraction via these muscles. Another consideration is significant gender differences in rodents with male skin approximately 40% thicker than females [106]. Rodent diabetic models are commonly used, and diabetes is easily induced in rodents. For chronic wounds, the major limitation of this model is diabetes is generally induced one to two weeks prior to wound introduction. This limits the time for chronic effects of diabetes to fully materialize [105]. Rabbit and porcine models have also been explored but are more cost prohibitive and have their own anatomical limitations [107,108].
Regulatory considerations for hydrogel dressings
The classification and regulation of wound dressing products largely depends on the components and the source of the dressing material as both impact safety and efficacy. Biologically passive hydrogel dressings are commonly classified as ‘devices’ for regulatory purposes while dressings loaded with analgesics, antibiotics, growth factors and other biologics are classified as ‘combinational devices’ by the FDA. In 21-CFR, wound dressings are described using three letter codes: KGN for dressing, wound, and collagen, FRO for dressing, wound, and drug, and MGP for dressing, wound and burn, and occlusive [109]. The safety of each is rated as class I, class II, or class III, with class I devices posing the lowest risk to patients. Each class has different requirements to demonstrate safety and adherence to regulatory standards. Products containing processed human tissues and/or cells are classified as low risk (PHS 361: HCT/Ps, human cells, tissues or cellular-based products) [110]. Table 1 shows the classifications of approved diabetic wound products into regulatory classes and the associated regulatory pathway.
Table 1: Classification and regulatory pathways for approved diabetic wound products.
A subset of hydrogel dressings contains anti-microbials as combination devices. These dressings typically undergo increased regulatory scrutiny because of the risk of the development of antimicrobial resistance due to overuse or misuse of the products. Criteria for determining the appropriate use of anti-microbial dressings are provided by the Infectious Disease Society of America (ISDA) and the American Society of Plastic Surgeons (ASPS) [111].
The regulatory requirements for developing products for chronic cutaneous ulcers and burn wounds are described in the FDA guidance Ulcer and Wounds-Developing 2006 in which pre-clinical requirements, clinical trial design, and standard wound care are specified [112]. Critical considerations include absorption studies for combination devices containing drug or biologic, local irritation, and immunological reactions. FDA has a separate guidance document for wound dressings containing poly(diallyl dimethyl ammonium chloride) additive into Class II (special controls) [113]. Table 2 summarizes the levels of considerations associated with the different classes of drugs.
Table 2: Considerations and risks associated with commonly used drugs in combinational products.
Conclusions
Hydrogel dressings have been a critical aide in the treatment of non-healing diabetic wounds for decades. They have provided significant clinical benefits for wound hydration, autolytic debridement, and facilitation of gas transfer. However, wound management alone is often insufficient to facilitate successful healing due to diabetes-related impairments of several healing pathways. In the past 5 years, pre-clinical research of advanced hydrogel systems has shown improvement in healing when delivering bio-active polymers, multiple growth factors, stem cells and epithelial cells. Despite this success, to date there is only one FDA approved product delivering a growth factor. Scarcity in novel approved products highlights the complexity of the problem, and the need to develop dynamic treatments which attack several aspects of the diabetic wound. Polymeric and self-assembling peptide hydrogels are well poised to form the basis of these advanced treatments and warrant continued research.
Conflict of Interest
The authors declare no conflicts of interest.
Data Availability
Data sharing is not applicable to this article as no new data were created or analyzed in this study.
Acknowledgements
This work was supported in part by National Institutes of Health grant R21 AI139828 and Department of Defense grant KC170026.
References
- Control CfD, Prevention (2020) National diabetes statistics report, 2020. Atlanta GA: Centers for Disease Control and Prevention US Department of Health and Human Services.
- Bruun C, Siersma V, Guassora A, Holstein P, de Fine Olivarius N (2013) Amputations and foot ulcers in patients newly diagnosed with type 2 diabetes mellitus and observed for 19 years. The role of age, gender and coâ?morbidity. Diabet Med 30: 964-972.
- Zhang P, Lu J, Jing Y, Tang S, Zhu D, et al. (2017) Global epidemiology of diabetic foot ulceration: a systematic review and meta-analysis. Ann med 49: 106-116.
- Hicks CW, Selvarajah S, Mathioudakis N, Sherman RL, Hines KF, et al. (2016) Burden of infected diabetic foot ulcers on hospital admissions and costs. Ann Vasc Surg 33: 149-158.
- Lloyd LL, Kennedy JF, Methacanon P, Paterson M, Knill CJ (1998) Carbohydrate polymers as wound management aids. Carbohydr Polym 37: 315-322.
- Mayet N, Choonara YE, Kumar P, Tomar LK, Tyagi C, et al. (2014) A comprehensive review of advanced biopolymeric wound healing systems. J Pharm Sci 103: 2211-30.
- Paul W, Sharma CP (2004) Chitosan and alginate wound dressings: A short review. Trends in Biomaterials and Artificial Organs pp: 18-23.
- Shah SA, Sohail M, Khan S, Minhas MU, de Matas M, et al. (2019) Biopolymer-based biomaterials for accelerated diabetic wound healing: A critical review. Int J Biol Macromol 139: 975-993.
- Kolarsick PAJ, Kolarsick MA, Goodwin C (2011) Anatomy and Physiology of the Skin. J Dermatol Nurses Assoc 3: 203-213.
- Murray RZ, West ZE, Cowin AJ, Farrugia BL (2019) Development and use of biomaterials as wound healing therapies. Burns Trauma 7.
- Boateng JS, Matthews KH, Stevens HNE, Eccleston GM (2008) Wound healing dressings and drug delivery systems: A review. Journal of Pharmaceutical Sciences 97: 2892-2923.
- Enoch S, Grey JE, Harding KG (2006) Recent advances and emerging treatments. BMJ (Clinical research ed) 332: 962-965.
- Zhao R, Liang H, Clarke E, Jackson C, Xue M (2016) Inflammation in Chronic Wounds. Int J Mol Sci 17: pp. 2085.
- Brem H, Tomic-Canic M (2007) Cellular and molecular basis of wound healing in diabetes. J Clin Invest 117: 1219-1222.
- Zubair M, Ahmad J (2019) Role of growth factors and cytokines in diabetic foot ulcer healing: a detailed review. Rev Endocr Metab Disord 20: 207-217.
- Berlanga-Acosta J, Schultz GS, Lopez-Mola E, Guillen-Nieto G, Garcia-Siverio M, et al. (2013) Glucose toxic effects on granulation tissue productive cells: The diabetics' impaired healing. Biomed Res Int 2013.
- Zhao G, Usui ML, Lippman SI, James GA, Stewart PS, et al. (2013) Biofilms and inflammation in chronic wounds. Adv Wound Care 2: 389-399.
- Obrenovich ME, Monnier VM (2005) Apoptotic killing of fibroblasts by matrix-bound advanced glycation endproducts. Sci Aging Knowledge Environ 2005: pe3.
- Balaji S, King A, Crombleholme TM, Keswani SG (2013) The role of endothelial progenitor cells in postnatal vasculogenesis: Implications for therapeutic neovascularization and wound healing. Adv Wound Care 2: 283-295.
- Petrelli A, Di Fenza R, Carvello M, Gatti F, Secchi A, et al. (2012) Strategies to reverse endothelial progenitor cell dysfunction in diabetes. Exp Diabetes Res 2012: 471823.
- Field CK, Kerstein MD (1994) Overview of wound healing in a moist environment. Am J Surg 167: S2-S6.
- Leaper DJ, Schultz G, Carville K, Fletcher J, Swanson T, et al. (2012) Extending the TIME concept: What have we learned in the past 10 years? Int Wound J 9: 1-19.
- Agrawal P, Soni S, Mittal G, Bhatnagar A (2014) Role of Polymeric Biomaterials as Wound Healing Agents. Int J Low Extrem Wounds 13: 180-190.
- Dyson M, Young S, Pendle CL, Webster DF, Lang SM (1988) Comparison of the effects of moist and dry conditions on dermal repair. J Invest Dermatol 91: 434-439.
- Sibbald RG, Williamson D, Orsted HL, Campbell K, Keast D, et al. (2000) Krasner D, et al. Preparing the wound bed--debridement, bacterial balance, and moisture balance. Ostomy Wound Managen 46: 14-22.
- Tuchscherr L, Korpos È, van de Vyver H, Findeisen C, Kherkheulidze S, et al. (2018) Staphylococcus aureus requires less virulence to establish an infection in diabetic hosts. Int J Med Microbiol 308: 761-769.
- Woodmansey EJ, Roberts CD (2018) Appropriate use of dressings containing nanocrystalline silver to support antimicrobial stewardship in wounds. Int Wound J 15: 1025-1032.
- Rustogi R, Mill J, Fraser JF, Kimble RM (2005) The use of Acticoat in neonatal burns. Burns 31: 878-882.
- Thomas J (2007) Treating a non-healing diabetic foot ulcer using Acticoat Moisture Control and the Lean improvement technique. WOUNDS UK 3: pp. 136.
- Yin HQ, Langford R, Burrell RE (1999) Comparative evaluation of the antimicrobial activity of ACTICOAT antimicrobial barrier dressing. The Journal of burn care & rehabilitation 20: 195-200.
- Penny HL, Webster N, Sullivan R, Spinazzola J, Green A, et al. (2009) The Use of Acticoat Moisture Control for the Successful Treatment of Diabetic Ulcers. Adv Skin Wound Care 22: 453-456.
- Falanga V (2005) Wound healing and its impairment in the diabetic foot. The Lancet 366: 1736-1743.
- Hermans MH (1987) HydroColloid dressing (Duoderm) for the treatment of superficial and deep partial thickness burns. Scandinavian journal of plastic and reconstructive surgery and hand surgery 21: 283-285.
- Kivman GY, Lyashenko YV, Rabinovich ÉZ, Fleiderman LI (1994) Hydrocolloidal dressings-A new generation of substances for the treatment of wounds and burns (review). Pharm Chem J 28: 630-637.
- Skorkowska-Telichowska K, Czemplik M, Kulma A, Szopa J (2013) The local treatment and available dressins designed for chronic wounds. J Am Acad Dermatol 68:e117-e126.
- Mir M, Ali MN, Barakullah A, Gulzar A, Arshad M, et al. (2018) Synthetic polymeric biomaterials for wound healing: a review. Prog Biomater 7: 1-21.
- Zheng Y, Liang Y, Zhang D, Sun X, Liang L, et al. (2018) Gelatin-Based Hydrogels Blended with Gellan as an Injectable Wound Dressing. ACS Omega 3: 4766-4775.
- Sood A, Granick MS, Tomaselli NL (2014) Wound dressings and comparative effectiveness data. Adv wound care 3: 511-529.
- Gupta A, Kowalczuk M, Heaselgrave W, Britland ST, Martin C, et al. (2019) The production and application of hydrogels for wound management: A review. Eur Polym J 111: 134-151.
- Kamoun EA, Kenawy ES, Chen X (2017) A review on polymeric hydrogel membranes for wound dressing applications: PVA-based hydrogel dressings. J Adv Res 8: 217-233.
- da Silva LP, Reis RL, Correlo VM, Marques AP (2011) Hydrogel-Based Strategies to Advance Therapies for Chronic Skin Wounds. Annu Rev Biomed Eng 21: 145-169.
- Sun G, Zhang X, Shen Y-I, Sebastian R, Dickinson LE, et al. (2011) Dextran hydrogel scaffolds enhance angiogenic responses and promote complete skin regeneration during burn wound healing. Proc Natl Acad Sci 108: 20976-20981.
- Flanagan M (1995) The efficacy of a hydrogel in the treatment of wounds with non-viable tissue. J Wound Care 4: 264-267.
- Li J, Mooney DJ (2016) Designing hydrogels for controlled drug delivery. Nat Rev Mater 1: 1-17.
- Abbasi M, Sohail M, Minhas MU, Khan S, Hussain Z, et al. (2019) Novel biodegradable pH-sensitive hydrogels: An efficient controlled release system to manage ulcerative colitis. Int J Biol Macromol 136: 83-96.
- Wong R, Ashton M, Dodou K (2015) Effect of Crosslinking Agent Concentration on the Properties of Unmedicated Hydrogels. Pharmaceutics 7: 305-319.
- Boontheekul T, Kong H-J, Mooney DJ (2005) Controlling alginate gel degradation utilizing partial oxidation and bimodal molecular weight distribution. Biomaterials 26: 2455-2465.
- Madl CM, Katz LM, Heilshorn SC (2018) Tuning bulk hydrogel degradation by simultaneous control of proteolytic cleavage kinetics and hydrogel network architecture. ACS Macro Letters 7: 1302-1307.
- George A, Shah PA, Shrivastav PS (2019) Natural biodegradable polymers based nano-formulations for drug delivery: A review. Int J Pharm 561: 244-264.
- Mogo??anu GD, Grumezescu AM (2014) Natural and synthetic polymers for wounds and burns dressing. Int J Pharm 463: 127-136.
- Chen RF, Wang CT, Chen YH, Chien CM, Lin SD, et al. (2019) Hyaluronic Acid-Povidone-Iodine Compound Facilitates Diabetic Wound Healing in a Streptozotocin-Induced Diabetes Rodent Model. Plast Reconstr Surg 143: 1371-1382.
- Thom SR, Hampton M, Troiano MA, Mirza Z, Malay DS, et al. (2016) Measurements of CD34+/CD45-dim stem cells predict healing of diabetic neuropathic wounds. Diabetes 65: 486-497.
- Hsu Y-Y, Liu K-L, Yeh H-H, Lin H-R, Wu H-L, et al. (2019) Sustained release of recombinant thrombomodulin from cross-linked gelatin/hyaluronic acid hydrogels potentiate wound healing in diabetic mice. Eur J Pharm Biopharm 135: 61-71.
- Edmonds M, Foster A (2000) Hyalofill: A new product for chronic wound management. Diabetic Foot 3: 29-30.
- Mayer P (2015) Hyaluronic acid gel improves wound healing in diabetic patients after digit amputations. The Mayer Institute, Hamilton, Ontario, Canada.
- Lei J, Chen P, Li Y, Wang X, Tang S (2017) Collagen hydrogel dressing for wound healing and angiogenesis in diabetic rat models. Int J Clin Exp Med 10: 16319-1627.
- He M, Sun L, Fu X, McDonough SP, Chu C-C (2019) Biodegradable amino acid-based poly (ester amine) with tunable immunomodulating properties and their in-vitro and in-vivo wound healing studies in diabetic rats’ wounds. Acta Biomater 84: 114-132.
- Jones A, Vaughan D (2005) Hydrogel dressings in the management of a variety of wound types: A review. J Orthop Nurs 9: S1-S11.
- Williams C (1994) Intrasite gel: A hydrogel dressing. British journal of nursing 3: 843-846.
- Wei L (2015) The application of moist dressing in treating burn wound. Open Med 10: 452-456.
- Ali L, Ahmad M, Aamir MN, Minhas MU, Rasul A, et al. (2019) Venlafaxine-loaded sustained-release poly(hydroxyethyl methacrylate-co-itaconic acid) hydrogel composites: their synthesis and in- vitro/in- vivo attributes. Iranian Polymer Journal 28: 251-258.
- Chiu Y-C, Cheng M-H, Engel H, Kao S-W, Larson JC, et al. (2011) The role of pore size on vascularization and tissue remodeling in PEG hydrogels. Biomaterials 32: 6045-6051.
- Dissemond J, Witthoff M, Brauns TC, Haberer D, Goos M (2003) pH-Wert des Milieus chronischer Wunden. Der Hautarzt 54: 959-965.
- Gianino E, Miller C, Gilmore J (2018) Smart Wound Dressings for Diabetic Chronic Wounds. Bioengineering (Basel) 5: pp.51.
- Bagherifard S, Tamayol A, Mostafalu P, Akbari M, Comotto M, et al. (2016) Dermal Patch with Integrated Flexible Heater for on Demand Drug Delivery. Adv Healthc Mater 5: 175-184.
- Holladay RJ, Christensen H, Moeller WD (2006) Treatment of humans with colloidal silver composition. US7135195B2.
- Masood N, Ahmed R, Tariq M, Ahmed Z, Masoud MS, et al. (2019) Silver nanoparticle impregnated chitosan-PEG hydrogel enhances wound healing in diabetes induced rabbits. Int J Pharm 559: 23-36.
- Ostrowska-Czubenko J, Gierszewska-Dru?¼y??ska M (2009) Effect of ionic crosslinking on the water state in hydrogel chitosan membranes. Carbohydrate Polymers 77: 590-598.
- Chen H, Cheng R, Zhao X, Zhang Y, Tam A, et al. (2019) An injectable self-healing coordinative hydrogel with antibacterial and angiogenic properties for diabetic skin wound repair. NPG Asia Materials11: 1-12.
- Chen H, Xing X, Tan H, Jia Y, Zhou T, et al. (2017) Covalently antibacterial alginate-chitosan hydrogel dressing integrated gelatin microspheres containing tetracycline hydrochloride for wound healing. Materials Science and Engineering C 70: 287-295.
- Overstreet D, McLaren A, Calara F, Vernon B, McLemore R (2015) Local gentamicin delivery from resorbable viscous hydrogels is therapeutically effective. Clin Orthop Relat Res 473: 337-347.
- Vasile BS, Oprea O, Voicu G, Ficai A, Andronescu E, et al. (2014) Synthesis and characterization of a novel controlled release zinc oxide/gentamicin-chitosan composite with potential applications in wounds care. Int J Pharm 463: 161-169.
- Yang K, Han Q, Chen B, Zheng Y, Zhang K, et al. (2018) Antimicrobial hydrogels: promising materials for medical applicationInt J Nanomedicine 13: 2217-2263.
- Dhivya S, Padma VV, Santhini E (2015) Wound dressings-A review. Bio Medicine 5: 22.
- Park JW, Hwang SR, Yoon IS (2017) Advanced Growth Factor Delivery Systems in Wound Management and Skin Regeneration. Molecules 22.
- ORTHO-McNEIL (2008) REGRANEX GEL 0.01% fda.gov: Food and Drug Administration.
- Dinh T, Braunagel S, Rosenblum BI (2015) Growth factors in wound healing: the present and the future? Clin Podiatr Med Surg 32: 109-119.
- Marti-Carvajal AJ, Gluud C, Nicola S, Simancas-Racines D, Reveiz L, et al. (2015) Growth factors for treating diabetic foot ulcers. Cochrane Database Syst Rev 10: CD008548.
- Pop M, Almquist B (2017) Biomaterials: A potential pathway to healing chronic wounds? Exp Dermatol 26: 760-763.
- Bladergroen BA, Siebum B, Siebers-Vermeulen KG, Van Kuppevelt TH, Poot AA, et al. (2009) In- vivo recruitment of hematopoietic cells using stromal cell-derived factor 1 alpha–loaded heparinized three-dimensional collagen scaffolds. Tissue engineering Part A 15: 1591-1599.
- Kim YH, Tabata Y (2017) Enhancement of wound closure by modifying dual release patterns of stromalâ?derived cell factorâ?1 and a macrophage recruitment agent from gelatin hydrogels. J Tissue Eng Regen Med 11: 2999-3013.
- Zhu Y, Hoshi R, Chen S, Yi J, Duan C, et al. (2016) Sustained release of stromal cell derived factor-1 from an antioxidant thermoresponsive hydrogel enhances dermal wound healing in diabetes. J Control Release 238: 114-122.
- Yang J, Van Lith R, Baler K, Hoshi RA, Ameer GA (2014) A thermoresponsive biodegradable polymer with intrinsic antioxidant properties. Biomacromolecules 15: 3942-3952.
- Tabata Y, Ikada Y (1999) Vascularization effect of basic fibroblast growth factor released from gelatin hydrogels with different biodegradabilities. Biomaterials 20: 2169-2175.
- Huang C, Orbay H, Tobita M, Miyamoto M, Tabata Y, et al. (2016) Proapoptotic effect of controlâ?released basic fibroblast growth factor on skin wound healing in a diabetic mouse model. Wound Repair and Regeneration. 24: 65-74.
- Yoo Y, Hyun H, Yoon S-J, Kim SY, Lee D-W, et al. (2018) Visible light-cured glycol chitosan hydrogel dressing containing endothelial growth factor and basic fibroblast growth factor accelerates wound healing in-vivo. Journal of Industrial and Engineering Chemistry 67:365-72.
- Jee J-P, Pangeni R, Jha SK, Byun Y, Park JW (2019) Preparation and in-vivo evaluation of a topical hydrogel system incorporating highly skin-permeable growth factors, quercetin, and oxygen carriers for enhanced diabetic wound-healing therapy. Int J Nanomedicine 14: 5449-5475.
- Liu H, Zhao Y, Zou Y, Huang W, Zhu L, et al. (2019) Heparin-poloxamer hydrogel–encapsulated rhFGF21 enhances wound healing in diabetic mice. The FASEB Journal 33: 9858-9870.
- Beenken A, Mohammadi M (2009) The FGF family: biology, pathophysiology and therapy. Nature reviews Drug discovery 8: 235-53.
- Liang Y, Kiick KL (2014) Heparin-functionalized polymeric biomaterials in tissue engineering and drug delivery applications. Acta biomaterialia 10: 1588-600.
- Freudenberg U, Zieris A, Chwalek K, Tsurkan MV, Maitz MF, et al. (2015) Heparin desulfation modulates VEGF release and angiogenesis in diabetic wounds. Journal of Controlled Release 220: 79-88.
- Roberts JJ, Farrugia BL, Green RA, Rnjak-Kovacina J, Martens PJ (2016) In situ formation of poly (vinyl alcohol)–heparin hydrogels for mild encapsulation and prolonged release of basic fibroblast growth factor and vascular endothelial growth factor. J Tissue Eng 7.
- Rustad KC, Wong VW, Sorkin M, Glotzbach JP, Major MR, et al. (2012) Enhancement of mesenchymal stem cell angiogenic capacity and stemness by a biomimetic hydrogel scaffold. Biomaterials 33: 80-90.
- Wu Y, Chen L, Scott PG, Tredget EE (2007) Mesenchymal stem cells enhance wound healing through differentiation and angiogenesis. Stem cells 25: 2648-2659.
- Xu Q, Sigen A, Gao Y, Guo L, Creagh-Flynn J, et al. (2018) A hybrid injectable hydrogel from hyperbranched PEG macromer as a stem cell delivery and retention platform for diabetic wound healing. Acta biomaterialia 75: 63-74.
- Kaisang L, Siyu W, Lijun F, Daoyan P, Xian CJ, et al. (2017) Adipose-derived stem cells seeded in Pluronic F-127 hydrogel promotes diabetic wound healing. Journal of Surgical Research 217: 63-74.
- Zhao L, Niu L, Liang H, Tan H, Liu C, et al. (2017) pH and glucose dual-responsive injectable hydrogels with insulin and fibroblasts as bioactive dressings for diabetic wound healing. ACS applied materials & interfaces 9: 37563-37574.
- Webber MJ, Appel EA, Meijer E, Langer R (2016) Supramolecular biomaterials. Nature materials 15: 13-26.
- Koch F, Müller M, König F, Meyer N, Gattlen J, et al. (2018) Mechanical characteristics of beta sheet-forming peptide hydrogels are dependent on peptide sequence, concentration and buffer composition. Royal Society open science 5.
- He B, Ou Y, Chen S, Zhao W, Zhou A, et al. (2017) Designer bFGF-incorporated d-form self-assembly peptide nanofiber scaffolds to promote bone repair. Materials Science and Engineering C 74: 451-458.
- Liu W, Wong-Noonan S, Pham NB, Pradhan I, Spigelmyer A, et al. (2019) A genetically engineered Fc-binding amphiphilic polypeptide for congregating antibodies in-vivo. Acta biomaterialia 88: 211-223.
- Pham NB, Liu W, Schueller NR, Gawalt ES, Fan Y, et al. (2019) Toward reducing biomaterial antigenic potential: a miniaturized Fc-binding domain for local deposition of antibodies. Biomaterials science 7: 760-772.
- Van den Broek LJ, Limandjaja GC, Niessen FB, Gibbs S (2014) Human hypertrophic and keloid scar models: principles, limitations and future challenges from a tissue engineering perspective. Experimental dermatology 23: 382-386.
- Nayak S, Dey S, Kundu SC (2013) Skin equivalent tissue-engineered construct: co-cultured fibroblasts/keratinocytes on 3D matrices of sericin hope cocoons. PLoS One 8: e74779.
- Sami DG, Heiba HH, Abdellatif A (2019) Wound healing models: a systematic review of animal and non-animal models. Wound Medicine 24: 8-17.
- Wong VW, Sorkin M, Glotzbach JP, Longaker MT, Gurtner GC (2011) Surgical approaches to create murine models of human wound healing. J Biomed Biotechnol 2011: 969618.
- Seaton M, Hocking A, Gibran NS (2015) Porcine models of cutaneous wound healing. ILAR journal 56: 127-138.
- Rittié L (2016) Cellular mechanisms of skin repair in humans and other mammals. Journal of cell communication and signaling 10: 103-120.
- Snyder D, Sullivan N, Schoelles K (2012) Skin substitutes for treating chronic wounds. Technology Assessment 2012: 290-2007.
- Dickinson LE, Gerecht S (2016) Engineered biopolymeric scaffolds for chronic wound healing. Front physiol 7: pp.341.
- FDA Executive Summary (2016) Classification of Wound Dressings Combined with Drugs.
- Ulcer CC (2006) Guidance for industry chronic cutaneous ulcer and burn wounds-developing products for treatment.
- FDA (2009) Guidance for Industry and FDA Staff: Class II Special Controls Guidance Document: Wound Dressing with Poly-(diallyl dimethyl ammonium chloride) (pDADMAC) Additive. US Department of Health and Human Services Food and Drug Administration Center for Devices and Radiological Health.